The term ‘Dendritic Cells’ (DCs) represents a family of immune cells derived from CD34+ hematopoietic stem cells in the bone marrow, with various functions that provide a key link between the innate and adaptive immune responses. The most widely described function of DCs is to capture, process, and present antigens to adaptive immune cells and mediate their transition to effector functions. In fact, DCs are the only antigen-presenting cells capable of stimulating naïve T-cells. In recent years, DCs have become the focus of translational research efforts to describe the role these cells play in allergies, autoimmunity, and cancer as well as their role in vaccine responses. In this blog, we explore the flow cytometry approaches used to examine DCs and their potential as therapeutic targets.
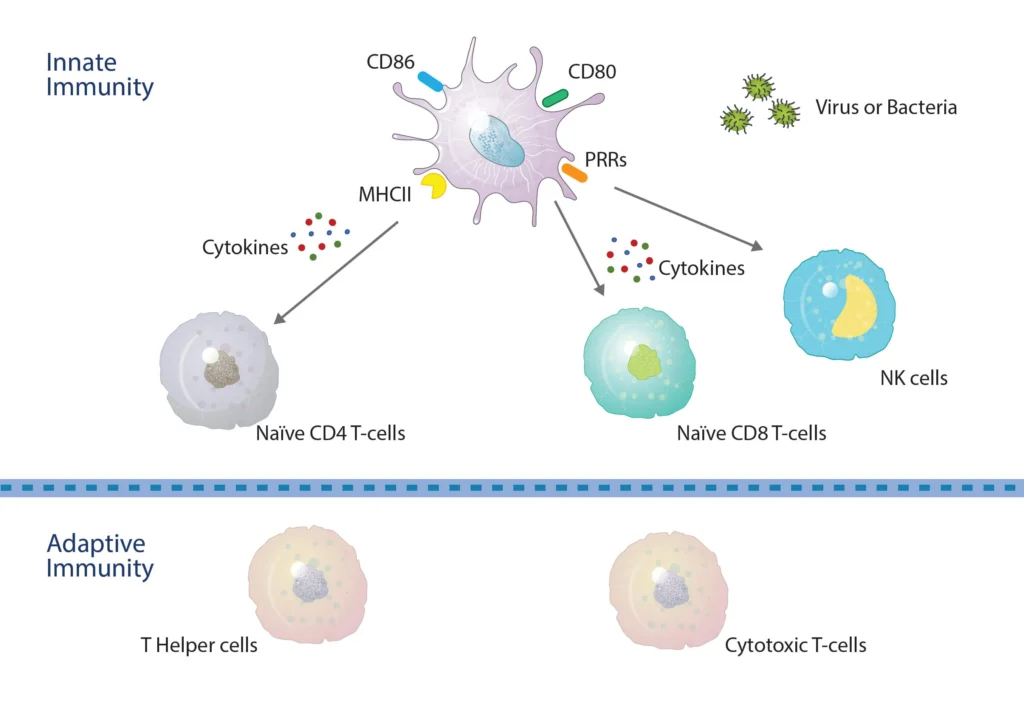
Figure 1. DCs bridge across both the innate and adaptive immune systems. Within the innate system, Pathogen-Associated Molecular Patterns (PAMPs) and Damage/Danger Associated Molecular Patterns (DAMPS) interact with toll-like receptors for the activation of DCs and the production of key cytokines that activate both NK and T-cells, resulting in CD4 and CD8 T-cell proliferation and differentiation. DCs internalize antigen peptides for presentation on Major Histocompatibility Complex molecules. Those loaded onto MHC II (HLA-DR) are recognized by CD4 T-cells, whereas those loaded onto MHC I (HLA-ABC) are recognized by CD8 T-cells.
The Life of DCs described through Flow Cytometry.
DCs are rare cells in peripheral blood and are typically identified by the exclusion of all other lineage markers (Lin-: including CD3, CD19, CD20, and CD56) associated with T, B, and NK cells along with monocytes and granulocytes. Immature DCs are located mainly in lymphoid and peripheral tissues where they capture antigens through an enhanced capacity for phagocytosis, and in response, undergo maturation that is associated with increased MHC I and II expression coupled with costimulatory molecule expression (CD80, CD86, and CD40), before re-localization to the lymphoid organs. The process of DCs maturation can be assessed using flow cytometry analysis of these markers. Fig. 2 shows representative flow cytometry analysis and gating strategies employed for DCs maturation assessment. For most research applications, DCs are generated in vitro using the prolonged (4-7 day) stimulation of peripheral blood monocytes with GM-CSF and IL-4. These so-called monocyte-derived DCs (Mo-DCs) can be matured by extended culture in the presence of microbial-, pro-inflammatory or T-cell-derived stimulatory molecules.
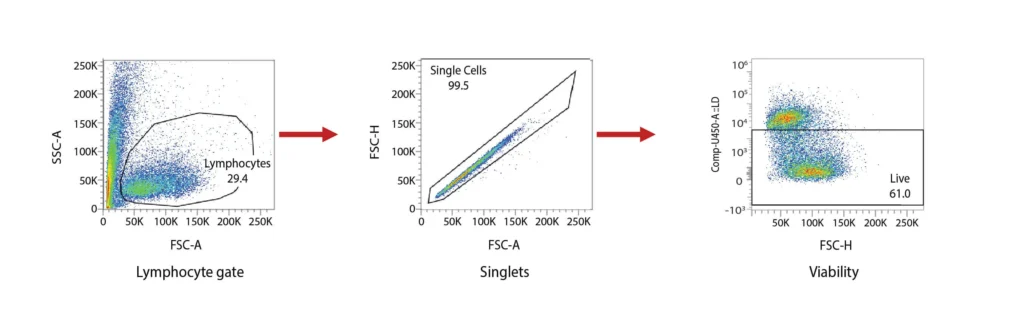
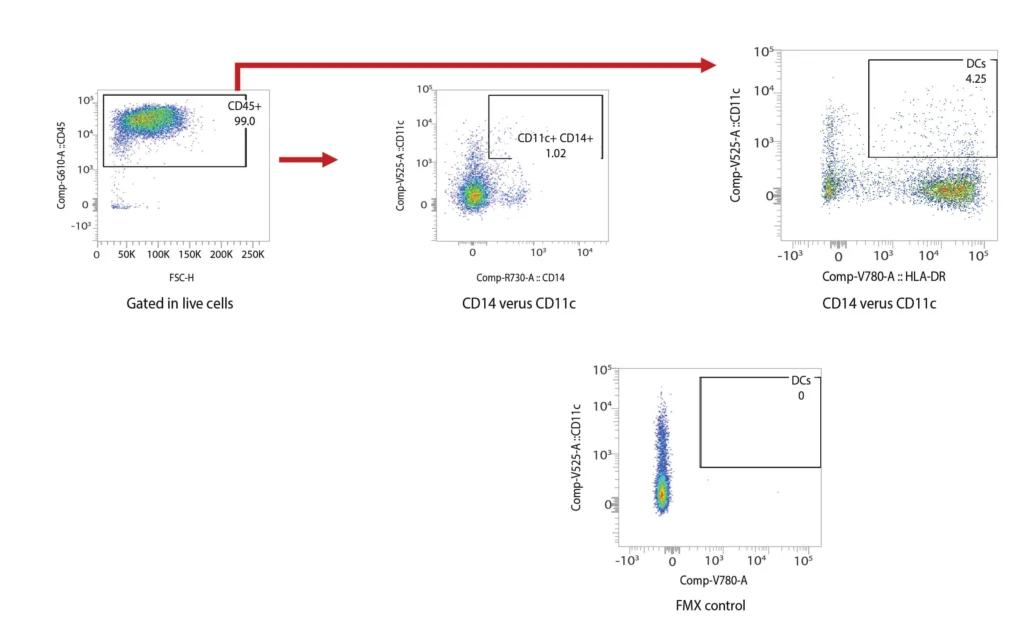
CD40
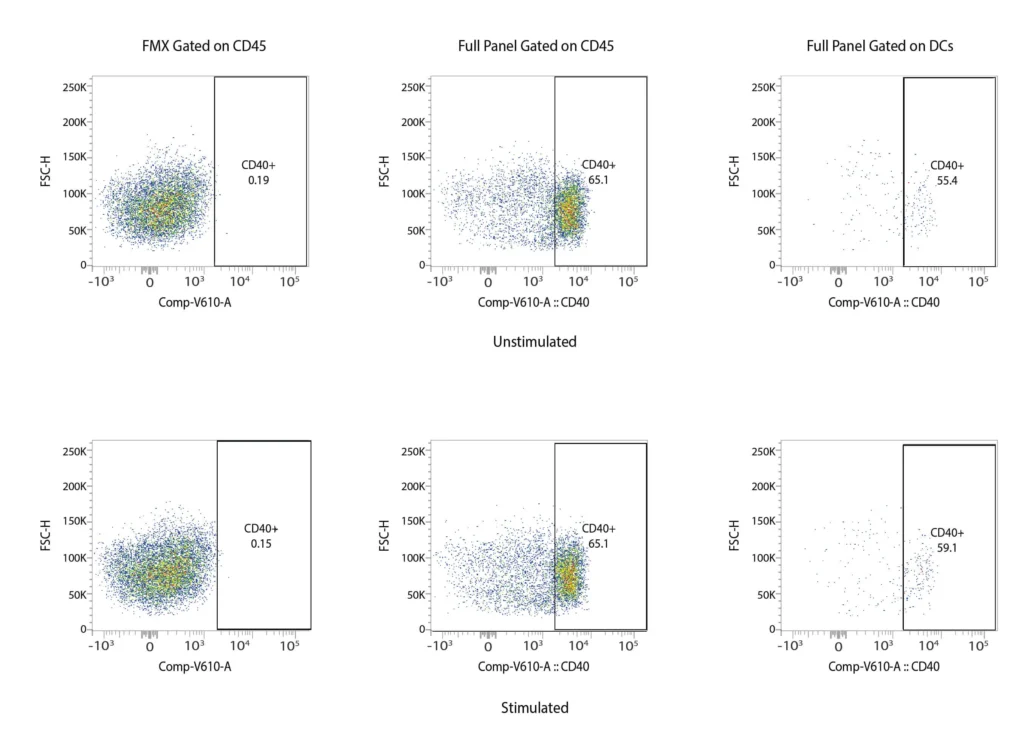
CD80
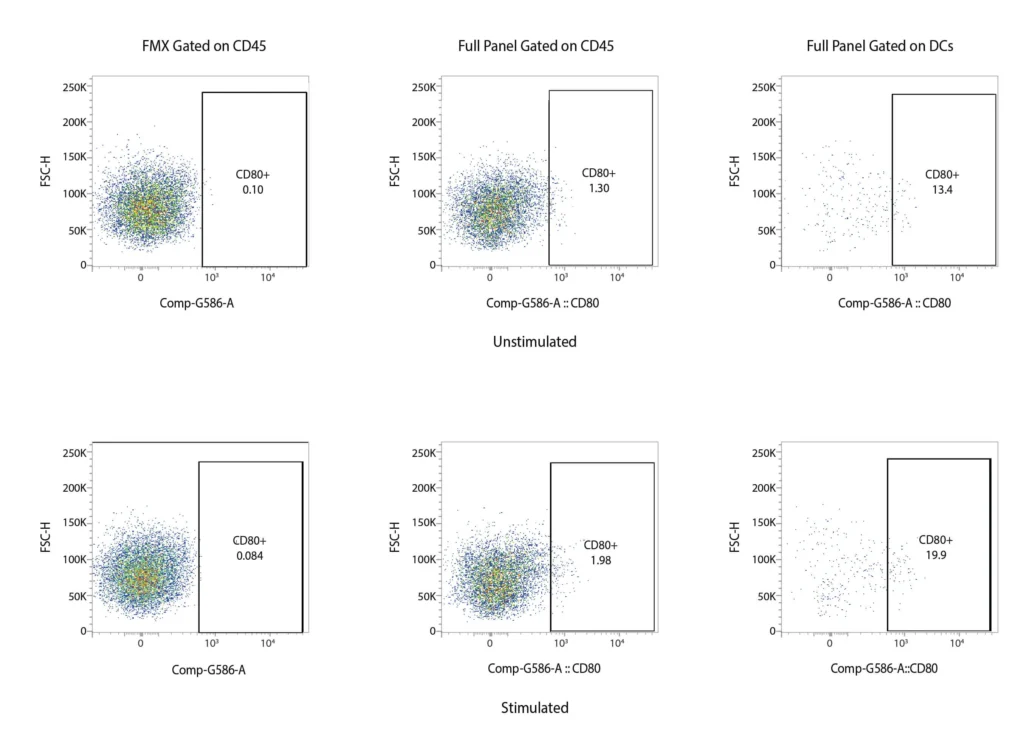
CD83
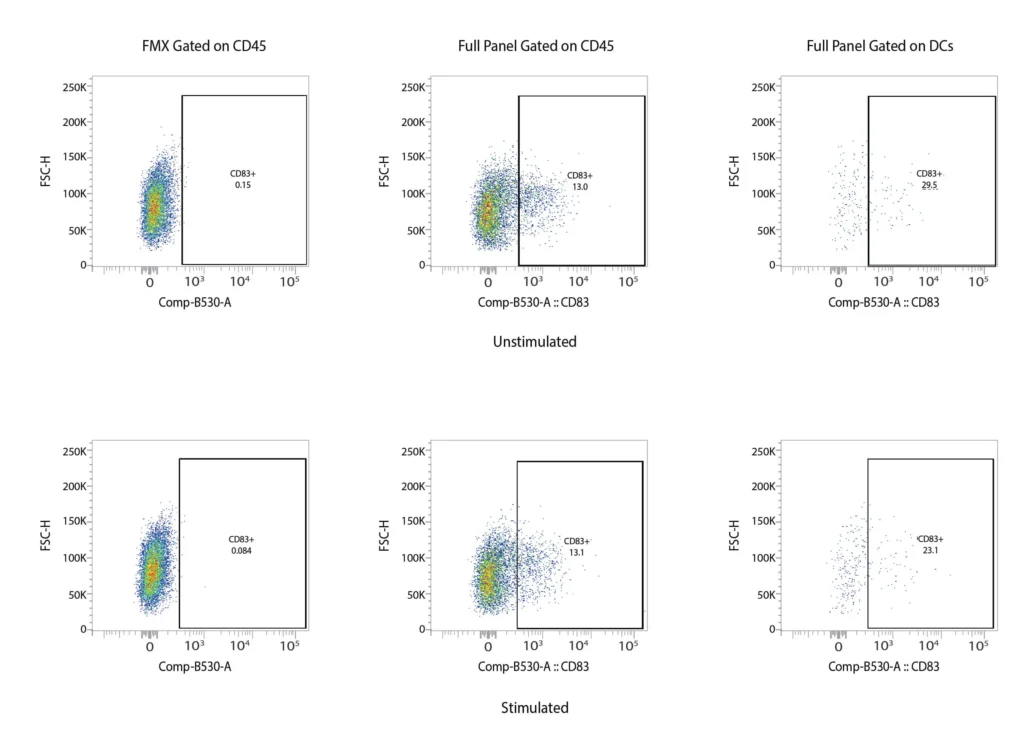
CD86
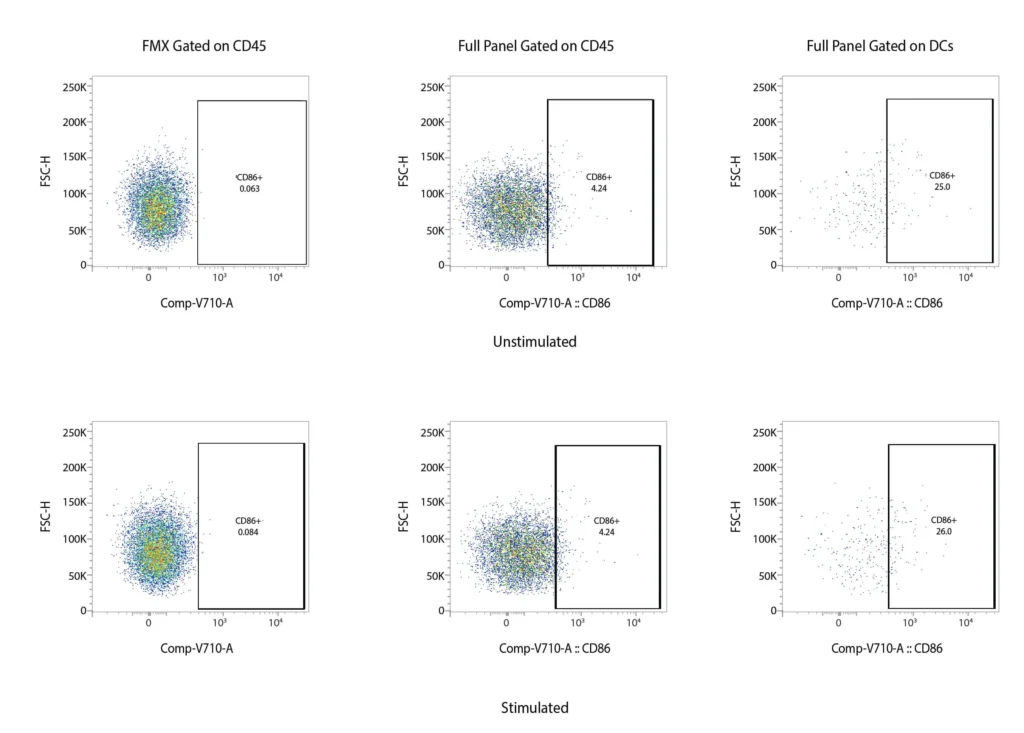
Figure 2. Representative flow cytometry data of DC profiling. Panel A outlines a gating strategy for myeloid DC profiling. Panels B (CD40), C (CD80), D (CD83), and E (CD86) demonstrate the analysis of activation markers for DC maturation.
Lineage of DCs
Whereas the origins and maturation of DCs are extensively studied in mice models, human DC origins, particularly in relation to monocytes is still an active area of research. A granulocyte, monocyte, and DC progenitor (GMPD) gives rise to both granulocytes and a monocyte/DC progenitor. Human DC progenitors and their subsequent developmental path can be monitored using flow cytometry, mainly using their lack of lineage and BDCA markers, coupled with CD45RA, CD117, CD115, C116, CD123, CD34, CD135 markers (see table 1).
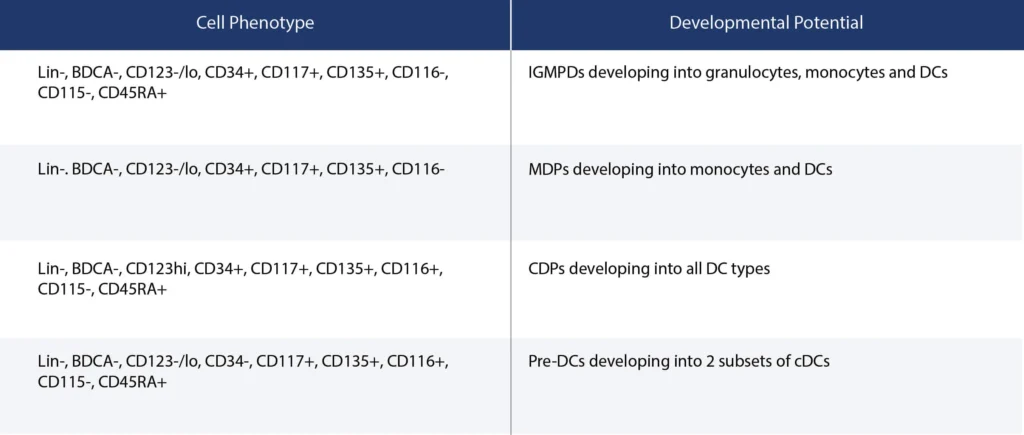
Table 1.Summary of markers used to track DC profiles using flow cytometry.
All DCs express high levels of MHC class II (HLA-DR) and so can be identified using flow cytometry by gating on class II HLA for further analysis. There are two main types of DCs- myeloid (mDCs) and plasmacytoid dendritic cells (pDCs). pDCs are similar to antibody-secreting plasma cells, although they have very unique secretory phenotypes. They arise from lymphoid progenitors and are found on lymph nodes, tonsils, the thymus, and spleen. pDCs typically lack myeloid antigens (CD11b, CD11c, CD13, and CD33) but express CD45RA, variable CD2, and CD7, and can be distinguished from mDCs by flow cytometry using their expression of CD123 (IL-3R), CD303 (BDCA-2), and CD304 (BDCA-4). Recent studies have demonstrated that virus-activated pDCs are able to induce the cytokine-driven maturation of TLR9-activated memory B cells into plasma cells, initially through IFN-induced differentiation into plasmablasts and then into antibody-secreting plasma cells via the activity of IL-6.
mDCs share markers in common with monocytes and granulocytes (CD13, CD33, CD16) but lack either CD14 or CD16, and have been described to form either CD1c+ or CD141+ populations. mDCs have tissue-associated counterparts. These Conventional or Classical Pre-cDCs differentiate out into two distinct types, cDC1 and cDC2 based on CD172 (SIRP-α) expression.
pDCs and cDCs are typically defined by their transcription factors- pDCs express both IFR4 and IFR8, whereas only cDC1 expresses IFR8 and only cDC2 expresses IFR4 (Collin and Bigley, 2018). cDC2 is the prominent population in both blood and in lymphoid tissue and express the myeloid markers CD11c, CD33, CD11b, CD13, and receptors TLR4, TLR6, TLR2/6, TLR8, and TLR9. The primary function of these cells is to protect against microbial infection through optimal CD4 T-cell priming to promote Th-17 and Th-2 immune responses. However, they are also capable of cross-presentation to CD8 T-cells, although this function is primarily covered by cDC1 cells that produce Type III interferon to induce and prime the CD8 T-cells to target tumors and intracellular pathogens. The functionally distinct receptors on cDC1 cells include TLR3, TLR9, and TLR10.
Therapeutic Applications of DCs
Since dendritic cells are ‘professional’ antigen-presenting cells that stimulate CD4 and CD8 T-cell responses, they are now being looked at as a potential mechanism for enhanced immune responses to tumors. The roles of DCs in cancer are very complex. DCs modulate the magnitude and duration of the infiltrating cytotoxic T-cell responses, however, due to the inherent plasticity of DCs, tumor-associated DCs are often dysfunctional. During tumor escape, regulatory DCs appear to play a critical role in the development of an immunosuppressive tumor microenvironment and contribute to tumor tolerance. Despite this, there is a growing body of evidence indicating that DCs play a central role in the efficacy of cancer immunotherapy. Even immunotherapies that are not directly associated with DC activities such as checkpoint blockade therapies and adoptive cell transfer therapies appear to be contingent on DC responses for efficacy. With the growing understanding of the role of DCs in antitumor immunity, researchers are now evaluating the potential of DCs for immunotherapeutic strategies.
DC-Based Cancer Vaccines
Crucial to the application of DCs as cancer vaccines is the concept of loading or pulsing DCs ex vivo with tumor-specific antigens. The first example of this approach was the DC-based vaccine Provenge (sipuleucel-T) for late-stage prostate cancer. This autologous process involves harvesting of DCs from the patient and pulsing with a fusion protein of prostate acid phosphatase (PAP) and GM-CSF. PAP is presented on most prostate cancer cells, whereas the cytokine GM-CSF helps to mature the DCs. Following pulsing ex vivo, the loaded DCs are infused back into the patient. Coupled with chemotherapy and monoclonal antibody treatment protocols, this was shown to extend patient life significantly. However, DC-based cancer-targeting is far from being a mature platform and subsequent generations of DCs therapy strategies are in development including combinations of loading, either with FLT3L or using RNA/DNA modification approaches (Benteyn et. al. 2014; Marte, B, 2020). To date, DC-based cancer vaccines have also been used to treat melanoma, urological-, brain, and lung tumors, resulting in significant tumor shrinkage and disease stabilization.
Processes for DC vaccination manufacturing vary widely in their methods of culturing, activation, maturation, and antigen loading but almost all DC vaccines have high safety and tolerance profiles. The challenges to the widespread adoption of DC-based cancer vaccines are similar to that of CAR-T cell approaches in that specific tumor characteristics and the immunosuppressive status of the tumor microenvironment may directly hamper the effectiveness of cellular-based therapies.
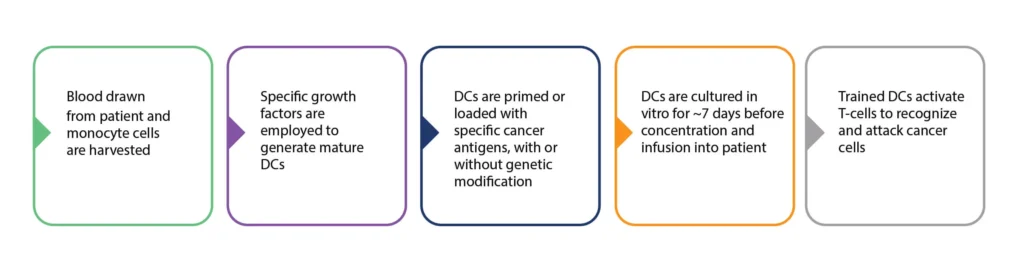
Figure 3. Summary of the process of DC cancer vaccine production. Personalized DC vaccine production involves the harvesting of patient monocytes, and the in vitro generation of mature DCs. These DCs are then primed/programmed and expanded before re-infusion into the patient. There are many variations in the processes employed at these various stages.
Response rates to DC vaccination vary from 10-15% based on the cancer type and the stage. This is significantly lower than that of immune checkpoint inhibition (ICI). Current clinical immunotherapies frequently include immune checkpoint inhibition- a process that targets immune checkpoint biomarkers such as CTLA-4, PD-1, and PDL-1, that are upregulated in certain cancers, and result in a tumor microenvironment resulting in the anergy of cytotoxic T-cells. Checkpoint inhibitors antagonize CTLA-4 and PD-1/PDL-1, to relieve the inhibition of T-cell activation and T-cell effector functions respectively. Importantly, the robust immune-inducing properties of DC vaccines make them highly suitable for combination therapy approaches, such as coupling DC vaccines with ICI, whereas their low toxicity profiles mean they may also have value as adjuvant to cancer (van Willigen et. al. 2018).
The Future of DC Cancer Vaccines
The success of DC-based cancer vaccines is dependent on overcoming several factors from the cost of such personalized treatments to the long-term survival of programmed DCs in patients and the maintenance of their migratory capacity. To this end, monitoring of patients during and post-treatment is going to be critical in determining the factors impacting the efficacy of this approach across different tumor profiles. Flow cytometry provides a powerful means to evaluate DC subsets in patients and identify phenotypic factors contributing to tumor suppression and the success of cellular therapeutics. Assessment of the activation and inhibitory molecules expressed on the surface of DCs along with their cytokine signatures provides a means of tracking patient responses to immunotherapeutic approaches to a variety of tumor types (Carenza et al 2021).
KCAS Bio is supporting many of these clinical DC initiatives with customized, validated, flow cytometry panels to examine DC profiles and their maturation markers along with cell sorting services for these rare cell populations for downstream phenotypic profiling.
References
- Collin, M., & Bigley, V. (2018). Human dendritic cell subsets: an update. Immunology, 154(1), 3–20. https://doi.org/10.1111/imm.12888
- PBenteyn, D. et. al. (2015) mRNA-Based dendritic cell vaccines. Expert Rev Vaccines 14(2):161-76. doi: 10.1586/14760584.2014.957684. Epub 2014 Sep 8
- Marte, B A Dendritic Cell Cancer Vaccine Nature Milestones Vaccines Nov. 2020 S21
- Van Willigen, W., et. al. (2018) Dendritic Cell Cancer Therapy: Vaccinating the Right Patient at the Right Time. Frontiers in Immunology. https://doi.org/10.3389/fimmu.2018.02265
- Carenza, C., et. al. (2021) Comprehensive Phenotyping of Dendritic Cells in Cancer Patients by Flow Cytometry. Cytometry Part A 99A: 218-230.