There is no question that the discovery of vaccines spearheaded the path of modern medicine and in so doing, eradicated at least two diseases, smallpox, and rinderpest from the global population. Today’s modern vaccines are being developed not only to tackle infectious diseases but also for the treatment and prevention of autoimmune diseases and cancers. Whereas vaccines for infectious diseases and cancer are designed to provoke a specific Th 1-driven immune response to target and reject the tumor or pathogen, vaccines driving Th 2 responses appear to be the best at targeting autoimmune diseases. Understanding the driving factors behind these underlying responses is central to the development of safe and effective vaccines, and flow cytometry provides unprecedented clarity on how the immune system responds to different vaccine strategies.
Never before in modern medicine, has there been such a global-wide initiative to fast-track the development of a vaccine as there is now with the current SARS-CoV-2 pandemic. But with so many different vaccine systems and formulations being developed, it is difficult to predict which ones will provide long term protection, or even how long will it take for us to know which are the safest and most effective against this new virus. The truth is that we still know surprisingly little about the immunological mechanisms and responses that are associated with the safest and most effective vaccines. There are many factors that come into play when developing a vaccine some of which are summarized in Fig, 1.
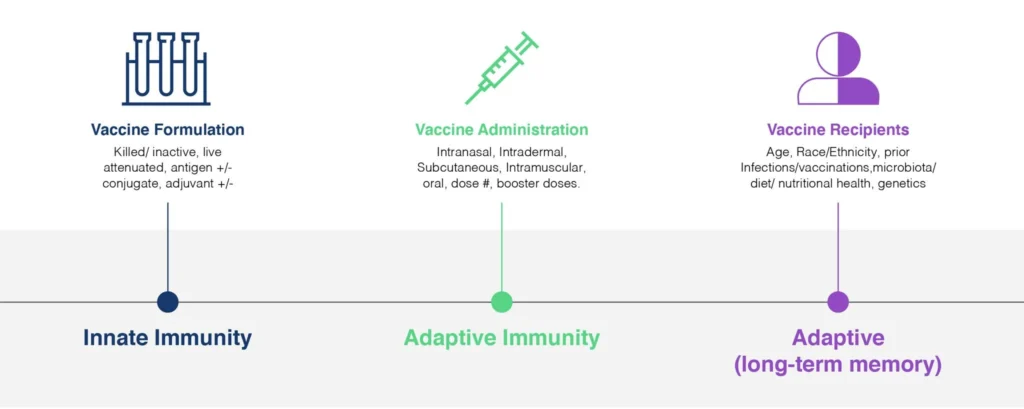
Fig. 1. Vaccine Efficacy is influenced by a diverse array of factors, from the design-, formulation- and dosing of the vaccine itself, to the profile of the recipients. An effective vaccine needs to invoke three distinct categories of the immune response: innate immunity, adaptive immunity, and immune memory. Flow Cytometry is uniquely able to provide valuable clinical read-outs all of these responses and is a powerful tool in the development of safe and effective vaccines addressing our current and future challenges.
So what makes a good vaccine?
First and foremost, a vaccine should be SAFE, even to individuals who are immuno-compromised. And whereas no vaccine is 100% safe, the acceptable levels of adverse events depend on the severity of the target disease and its perceived impact on the population.
Any approved vaccine should also display high efficacy, and optimally induce a neutralizing immunity- i.e. more complete protection from infection. But this is quite complicated and there are many facets to this efficacy.
There are also practical considerations, for a global Pandemic such as SARS-CoV-2, the cost of the vaccine is going to have a profound impact on its use. The WHO recommends that vaccines should cost less than 50 cents/dose for global use in developing countries, and vaccine formulations displaying high thermostability are needed for use in tropical environments. Oral administration via mucosal surfaces is preferred over parenterally, but this isn’t a widely applicable format. Effective vaccines should provide long-lived immunity. Some attenuated viruses give long-lived immunity after one or two doses. However, it is common for vaccines to require a course of doses with an adjuvant to achieve optimal efficacy.
What are clinical endpoints of Vaccine Efficacy?
Vaccine efficacy can be described as a number of different ways
Humoral Response-Frequency (titer) of humoral response is the most commonly used because of its relative simplicity. ELISA is the Gold Standard method of detecting antibodies (IgM, and IgG) targeting vaccine antigens. A higher antibody titer is associated with a more robust immune response to vaccination.
Establishment of Cellular Immunity and Memory is determined through the analysis of antigen-specific T-cells, and T-cell memory profiles (CD45RA, CCR7, CD27, CD28).
Cell Proliferation CFSE proliferation assays provide a readout of the vaccine’s immunogenicity.
CD8+ Cytotoxic Activity- CD8+ cytotoxic T-cell activity. This is typically measured by examining degranulation and the cytotoxic potential associated with CD107a, Granzyme, Perforin expression.
Cytokine and Chemokine Responses- cytokine responses to vaccination are critical for the induction and maintenance of appropriate humoral responses via potent B cell responses and dendritic cell activation.
Collectively, these characteristics can provide a window into a vaccine’s protective activity in a population. Flow cytometry represents a robust method to qualify and quantify many different immunological responses to vaccination, that can provide a valuable assessment of the short and long-term immunity provided by different vaccine types and formulations, administered through different vaccination protocols.
Clinical Applications of Flow Cytometry to Support Vaccine Development
Immunophenotyping
Flow Cytometry based Immunophenotyping provides a means of describing and enumerating different cell types, sub-classes, and functionalities within a sample. Monitoring the frequency of different immune cell populations and the differentiation and activation status of specific subsets (e.g. monocytes, NK T-cells, NK cells, CD4+ and CD8+ T-cells, Tregs, γδT cells, B-cells, plasmablasts) is essential to understanding the immunogenicity and efficacy of a vaccine.
Upon vaccine exposure, naïve T-cells (CD45RO) differentiate into central memory (TCM) (CD45RA, CCR7) within the lymphoid tissues and effector memory (TEM) (CD45RA, CD62L) within peripheral tissues. Examples of memory T-cells providing vaccine-induced immunity are numerous, however, the length of this memory varies considerably between vaccines.
The monitoring of activation markers (e.g. HLA-DR, CD38, Ki-67, BcL-2) on different immune cell populations can provide key insights into the kinetics of an immune response to vaccination, and provide clinical profiles for the stratification of patients and the determination of boosting requirements.
To date, no vaccine in human clinical trials has demonstrated protective efficacy through T-cell immunity alone.
Intracellular Cytokine Staining (ICS) to Measure Vaccine-Induced T-cell Immunogenicity
The quantification of antigen-specific CD4+ and CD8+ T cells in response to viral antigen challenge is a key measure of vaccine immunogenicity. Unlike ELISpot, ICS enables multiple cytokines to be measured in specific cell types. IFN-γ, IL-2, and TNF-α are the most profiled by ICS. However, with a carefully tailored panel design, it is possible to measure 12 or more different cytokines and chemokines within CD4+ and CD8+ T cells. This type of analysis can be critical to identify the features and qualities of a vaccine formulation that correlate with vaccine efficacy.
Activation-Induced Marker (AIM) Assays
This is performed in a very similar way to ICS, however rather than staining for intracellular cytokines, T-cell receptor-induced-surface markers such as PD-1, CD25, and OX40 are measured in response to vaccination, and can differentiate CD4+ and CD8+ T-cell responses. However, AIM assays appear to detect more CD4+ subtypes including Tregs and NK-T cells compared with ICS, which primarily detects CD4+ TEM. AIM studies have been shown to be valuable in vaccine development studies to detect novel memory T-cell responses that might be challenging to enumerate using ICS alone (Reiss et. al. 2017).
Quantitation of Antigen-Specific T cells
The quantitation of antigen-specific T-cells is an important characteristic in the evaluation of vaccine efficacy. The most recent methods employ tetramers – synthetic structures made from HLA molecules (four or more) linked together to form a multimeric complex that presents the antigen peptides to the immune system. First developed in the 1990s, these have become valuable tools in vaccine development and performance assessment. The tetramer constructs are engineered to bind to the T-cell receptors of the antigen-specific cells will high avidity, enabling them to be detected via the fluorescently attached probes. This strategy is particularly powerful in identifying and enriching rare cell populations, such as naïve antigen-specific T-cells, as well as enable an effective enumeration of CD8+ and CD4+ antigen-specific T-cells following vaccination (Fig. 2). A downside to using this methodology is that the tetramer complexes are both HLA- and antigen-epitope specific, and as such tetramer design requires knowledge of the HLA type of the subject being studied as well as considerable analysis of the major pathogen epitopes recognized by human systems that are represented in the vaccine. There are several algorithms available to identify immunogenic peptide regions that are most likely to be conserved within a pathogen, as well as a commercially available mutated form of the HLA alpha 3 domain that is able to reduce non-specific binding for class I binding to CD8+ T-cells (Jansen et. al. 2018).
The tetramer methodology still represents the only method to detect and enumerate antigen-specific CD4+ and CD8+ T-cell ex vivo and can be adapted to provide an effective means of cell sorting.
Fig. 2. TCRs recognize and bind to complexes expressed on the surface of antigen-presenting cells. These complexes are composed of MHC molecules and specific, antigen-derived peptides. Monomeric MHC/peptide complexes display low affinity to TCR and are therefore unstable; however, chemically coupled tetramers of these complexes maintain stable binding to multiple TCR. Labeling of the MCH tetramers with fluorescent molecules such as allophycocyanin (APC) or phycoerythrin (PE), allows us to detect antigen-specific T-cells by flow cytometry.
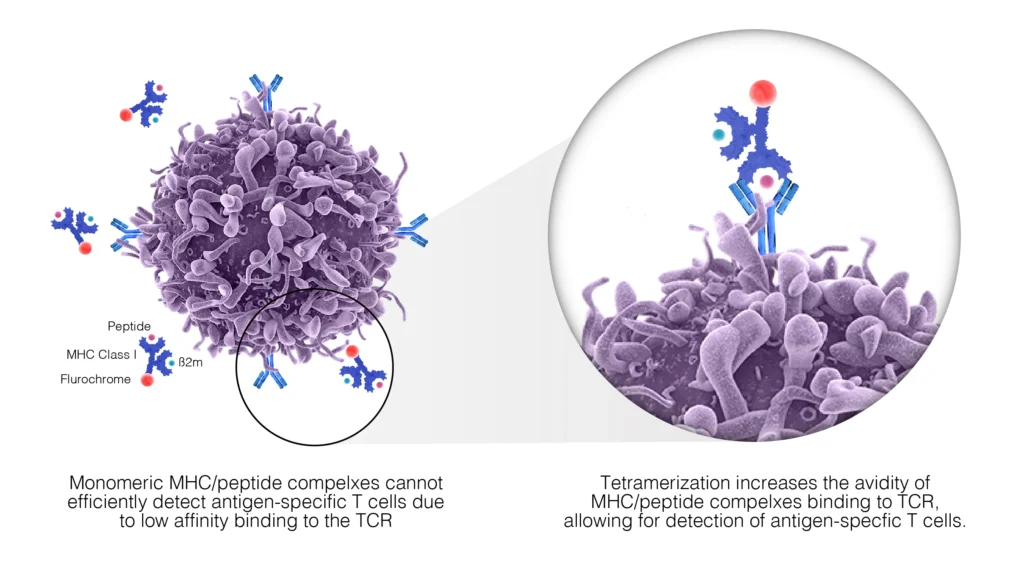
Cytotoxic CD8+ cells Following TCR engagement with peptide-MHC class I complexes, CD8+ cells exhibit several effector functions that can be measured with flow cytometry such as cytokine production (ICS) and cytotoxicity through the release of cytolytic granules containing Perforin and Granzymes, or through Fas/FasL interactions.
During the process of degranulation, preformed cytolytic granules consisting of lysosomes encapsulated with lysosomal-associated membrane proteins (LAMPs) fuse with the plasma membranes of the activated CD8+ T cells, to release the cytotoxic payload into the target cell. Since LAMPs are only present on the cell surface of T-cells undergoing degranulation, they have become a biomarker for CD8+ T-cell activity.
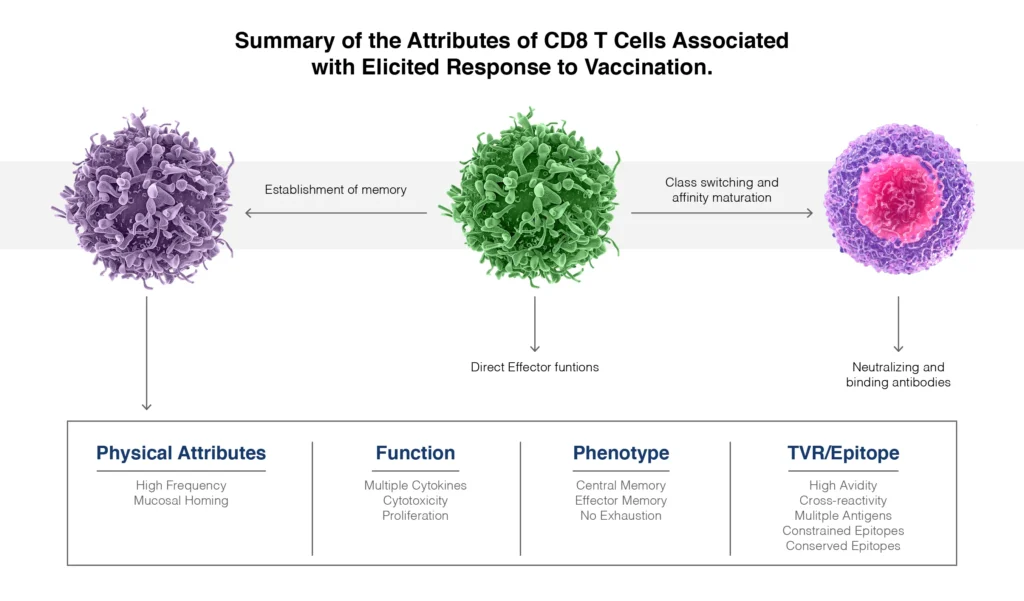
T-cell priming and B-cell activation. CD40L is expressed on the surface of activated CD4+ T cells and is one component of the helper interaction with B cells and CD8+ T cells. CD40L can be detected by two methods, either intracellular staining or by co-culture. B-cell engagement through transient expression of CD40L (CD154) on T-cell under inflammatory conditions results in the development of adaptive immunity. Studies have shown that CD40L is a potent adjuvant that is able to enhance the magnitude and polyfunctionality of CD4+ and CD8+ T-cell responses towards the Th 1 phenotype. These are associated with enhanced protective efficacy of traditional vaccines (Darrah et. al., 2007; Kannanganat et. al. 1996; Marzo et. al. 2004; Scriba et. al. 2010).
T-cell Polyfunctionality
Polyfunctionality analysis describes the response based on the number of functions (e.g. cytokine or chemokine release) per cell. This type of analysis can be challenging and difficult to interpret so frequently the readout is provided as the proportion of responding cells producing one-, two-, three functions without necessarily specifying these functions independently. This is commonly referred to as the degree of functionality. Higher polyfunctionality is associated with increased vaccine efficacy profiles with respect to the capacity of T-cells to kill target cells in vitro and control infection in vivo, providing increased protective immunity (Rossi et al. 2018).
ICS, as well as proteomic screening such as the Isolight™ system from Isoplexis™, can provide a view into the polyfunctionality of T-cells after vaccination, and studies are now clearly revealing a strong correlation between polyfunctionality and vaccine-induced, durable protective immunity (Zhou et. al. 2017).
Proliferation
The gold standard for cell proliferation assays involved the release of radioactive 51Cr. Although this is reliable, the use of radiolabelled isotopes is impractical for many laboratories. The use of flow cytometry for this application avoids this issue and provides a mechanism for measuring cytotoxicity at the cellular level. The analysis of proliferative responses to target antigens is made possible through the use of tracking dyes. The combination of different proliferation tracking dyes (general protein &/or membrane-labeling dyes) with other phenotypic and viability probes, can be used to provide powerful insights into cytotoxic activity and regulatory T-cell functions in response to vaccination or antigen challenge (Fig. 4).
There are careful considerations to take into account though including:
- the ability to maintain a bright signal from the specific probe without impacting cellular processes or proliferative potential via cytotoxicity,
- the overall stability of the probe over the timeframe of the assay,
- the spectral profile of the complete experimental system.
Carboxyfluorescein succinimidyl ester (CFSE) is a commonly used dye for proliferation assessment. Cells are labeled with CFSE and cultured in vitro during stimulation over several days. The dye is progressively diluted during cell proliferation. However, this has limited applications for the measurement of vaccine-induced T-cells, which may be at very low frequency within the sample. Careful controls should be implemented, including positivity controls using anti-CD3/CD28.
In addition, Ki-67 has become a widely studied biomarker for cell proliferation. Ki-67 is a nuclear antigen that is only expressed in recently or actively dividing cells. Intracellular staining for flow cytometry analysis Ki-67 expression has now formed the basis of many proliferation assays (Stubbe et. al. 2006).
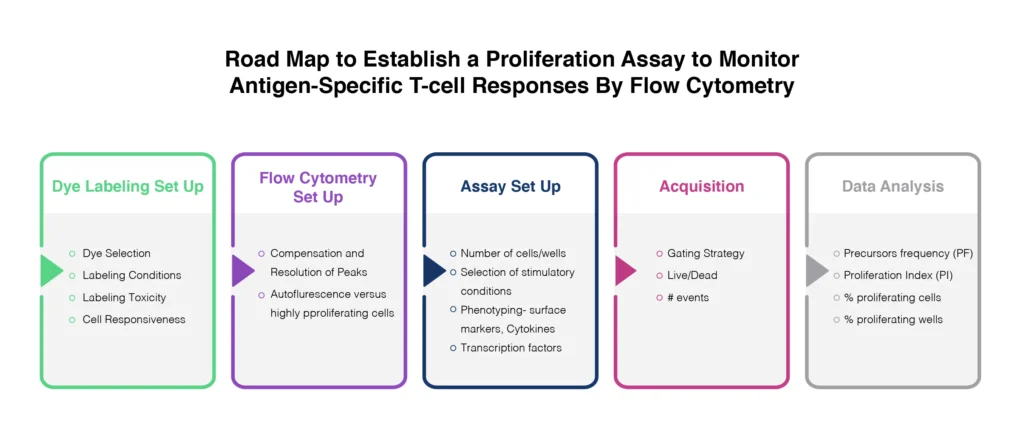
Plasmablast-Derived Polyclonal Antibody Responses
As previously mentioned, the go-to measurement of antibody responses to vaccination uses ELISA to determine antigen-specific antibody titers. These are primarily generated within the bone marrow and don’t necessarily represent the entire vaccine-induced repertoire of B-cell responses. After vaccination, activated B cells differentiate into plasmablasts in the lymphoid tissues, after which they exit into the circulation to target tissues where they mature into plasma cells. Typically, within 1-2 weeks after vaccination, a transient profile of plasmablasts can be identified in the bloodstream using flow cytometry-based biomarker analysis (Table 1). The frequency of vaccine-specific plasmablasts during this window, provides a unique insight into the overall B-cell response to the vaccine, without any interference from pre-existing cross-reactive antibodies that might be circulating in the serum. This strategy enables researchers to assess antibody avidity based on antigen-specific binding and antibody quantification (He et. al. 2011). Data from various vaccine studies show a large variability in acute plasmablast counts between individuals following vaccination, indicating that the plasmablast response is governed by multiple factors. A study of the H1N1 vaccine indicated that a plasmablast response at day 7 was predictive of increased antibody titers and of the establishment of immunological memory and enhanced immunity (Querec et. al. 2009).
Marker |
Function |
Memory B Cells |
Plasmablasts |
Plasma Cells |
CD19 |
Transmembrane signaling molecule, BCR complex component regulating signaling |
+ |
LOW |
LOW |
CD20 |
Transmembrane signaling molecule, BCR complex |
+ |
– |
– |
CD27 |
TNF receptor family |
+ |
++ |
+++ |
CD38 |
Signaling molecule and enzyme catalyzing formation of cyclic ADP-ribose |
+/- |
++ |
+++ |
CD138 |
Heparan sulfate proteoglycan |
– |
+/- |
+ |
Ki-67 |
Nuclear protein expressed during cell division |
– |
+ |
+/- |
Table 1. Flow Cytometry Markers of vaccine-induced plasmablasts and plasma cells.
Antibody-Dependent Cellular Cytotoxicity (ADCC)
Most vaccine development initiatives focus on inducing broad and potent cytotoxic T lymphocyte and Neutralizing Antibody responses that help to establish immunity. However, non-neutralizing antibodies may display broader cross-reactivity and still provide protection though mechanisms such as ADCC. ADCC is essentially FcγRIIIa (Cd16)-triggered degranulation resulting in target cell killing. The profiling of ADCC responses to post vaccinations can help evaluate the protective potential of the antibody response to the vaccine. Flow cytometry methods utilize a combination of membrane dyes such as PKH-26 and CFSE. Target cells in the samples are differentiated from effector cells by PKH-26 staining, and target cell killing is measured by the loss of CFSE staining from cells with compromised surface membranes (detected as PKH-26+/CFSE-). NK cells can be distinguished from monocytes in this analysis by using CD107a staining (Pollara et. al. 2018).
Final Thoughts
There are important factors to consider when assessing vaccine responses in vitro. Optimal timepoints for evaluation must be determined, and careful controls implemented. The correlation of vaccine efficacy with multiple clinical endpoints is essential to understanding the interaction of the vaccine components with the immune system. This analysis can help ascertain the functional characteristics of the vaccine that invoke a strong immune response, as well as highlight any risks associated with its widespread use.
The twentieth century saw a huge increase in the number of approved vaccines targeting both bacterial and viral infections, and this second decade of the twenty-first century has been designated the “Decade of Vaccines”. One aspect of this is the understanding that we need vaccines to induce CD4+ and CD8+ T cells in sufficient frequency and desired phenotype to support both the B-cells that spearhead the antibody response, but also to direct pathological clearance via cell-mediated effector functions.
For the current SARS-CoV-2 pandemic, the promise of a vaccine may seem a long way off for some. However, with the expedited clinical trial path now assessing 20+ different vaccine formations targeting the virus, many have raised concerns about the short and long-term safety of these vaccines. These concerns are not unfounded given that this virus appears to play havoc on the inflammation and immune system responses of some infected individuals. The potential risks of SARS-CoV-2 vaccines include Vaccine Associated Enhanced Respiratory Disease (VAERD) caused by a Th 2 biased immune response, as well as Antibody-Dependent Enhancement (ADE) through Fc-mediated viral entry resulting in innate macrophage activation. Both conditions can be fatal, and so comprehensive immune monitoring of the patients enrolled in these expedited clinical trials, using validated flow cytometry analysis, is one of the most robust ways we have right now to assess these risks, before the utilization of these vaccines across the globe.
Multiparameter flow cytometry is becoming increasingly used to assess immunogenicity in clinical trials for vaccines, particularly where T-cell responses represent a major component of efficacy. The development and validation of an increasing number of flow cytometry protocols to interrogate multiple immune system mechanisms allow us unprecedented insights into vaccine safety and efficacy.
References:
Darrah PA, Patel DT, De Luca PM, Lindsay RW, Davey DF, Flynn BJ, Hoff ST, Anderson P, Reed SG, Morris SL, Roederer M, Seder RA. (2007) Multifunctional TH1 cells define a correlate of vaccine-mediated protection against Leishmania major. Nat Med. 13(7):843–50.
Hem X-S., et. al. (2012) Plasmablast-Derived Polyclonal Antibody Response fter Influenza Vaccination. J. Immunol. Methods. Feb 28: 365(1-2):67-75
Jansen et al. (2018) Flow Cytometric Clinical Immunomonitoring Using Peptide–MHC Class II Tetramers: Optimization of Methods and Protocol Development. Front. Immunol. 9:8 doi 10.3389
Kannanganat S, Ibegbu C, Chennareddi L, Robinson HL, Amara RR. (1996) Multiple-cytokine-producing antiviral CD4 T cells are functionally superior to single-cytokine-producing cells. J Virol. 81(16):8468–76.
Marzo AL, Vezys V, Klonowski KD, Lee SJ, Muralimohan G, Moore M, Tough DF. Lefrancois L. (2004) Fully functional memory CD8 T cells in the absence of CD4 T cells. J Immunol. 173(2):969–75.
Pollara et al.,J. Pollara, C. Orlandi, C. Beck, R.W. Edwards, Y. Hu, S. Liu, S. Wang, R.A. Koup, T.N. Denny, S. Lu, G.D. Tomaras, A. Devico, G.K. Lewis, G. Ferrari. (2018) Application of area scaling analysis to identify natural killer cell and monocyte involvement in the GranToxiLux antibody-dependent cell-mediated cytotoxicity assay. Cytometry A, 93A pp. 436-447
Querec, T. D., Akondy, R. S., Lee, E. K., Cao, W., Nakaya, H. I., Teuwen, D., Pirani, A., Gernert, K., Deng, J., Marzolf, B., Kennedy, K., Wu, H., Bennouna, S., Oluoch, H., Miller, J., Vencio, R. Z., Mulligan, M., Aderem, A., Ahmed, R., and Pulendran, B. (2009). Systems biology approach predicts immunogenicity of the yellow fever vaccine in humans. Nat. Immunol. 10, 116–125.
Reiss S., Baxter A.E., Cirelli K.M., Dan J.M., Morou A., Daigneault A., Brassard N., Silvestri G., Routy J.P., Havenar-Daughton C., et al. (2017) Comparative analysis of activation-induced marker (aim) assays for sensitive identification of antigen-specific CD4+ T cells. PloS ONE. 12:e0186998. doi: 10.1371/journal.pone.0186998.
Rossi, J., et al. (2018) Preinfusion polyfunctional anti-CD19 chimeric antigen receptor Y cells associated with clinical outcomes in NHL. Blood Aug 23; 132 (8):804-814. https://www.ncbi.nlm.nih.gov/pmc/articles/PMC6107882
Scriba TJ, Tameris M, Mansoor N, Smit E, van der Merwe L, Fatima I, Keyser A, Moyo S, Brittain N, Lawrie A, Gelderbloem S, Veldsman A, Hatherill M, Hawkridge A, Hill AVS, Hussey GD, Mahomed H, McShane H, Hanekom WA. (2010) Modified vaccinia Ankara-expressing Ag85A, a novel tuberculosis vaccine, is safe in adolescents and children and induces polyfunctional CD4+ T cells. Eur J Immunol. 40(1):279–90.
Stubbe M, Vanderheyde N, Goldman M, Marchant A. (2006) Antigen-specific central memory CD4+ T lymphocytes produce multiple cytokines and proliferate in vivo in humans. J Immunol. 177(11):8185–8190. [PubMed: 17114495]
Zhou J, et al. (2017) CD8+ T-cell mediated anti-malaria protection induced by malaria vaccines; assessment of hepatic CD8+ T cells by SCBC assay. Human Vaccines & Immunotherapeutics 13: 1625-1629.